3D Bioprinting of Tissues and Organs
- Lucy Chen
- Nov 30, 2020
- 10 min read
Updated: Jul 15, 2023
Organ and tissue transplantation involves the replacement of a damaged organ or tissue with new, functioning tissue. Currently, most transplanted material comes from people other than the patient themselves, known as an allograft. In cases where material from the patient has been transplanted back into the patient, for example, to treat wounded skin, the process is known as an autograft. Transplants have been key treatments for many severe, rare, and end-stage diseases, such as diabetes, cystic fibrosis, and acute myeloid leukaemia (AML). These conditions can have genetic or infectious origins and many people die if they do not have a transplant. In the US, around 20 people a day die whilst waiting for an organ donation.
Many organs can be transplanted according to different procedures, but the key requirement for this is that the patient has a matching donor. The biology behind this lies in the immune system and how the body distinguishes between its own cells (the self) and invading cells, such as disease-causing viruses or bacteria (foreign).
The immune system is based on this recognition process, which is driven by antibodies and antigens. Antigens are proteins that may be able to generate an immune response, and antibodies are proteins that are complementary to antigens, which means they bind to antigens. This triggers a series of chemical reactions that leads to an immune response, causing the body to attack the cells that are attached to that specific antigen.
The immune system is based on this recognition process, which is driven by antibodies and antigens. Antigens are proteins that may be able to generate an immune response, and antibodies are proteins that are complementary to antigens, which means they bind to antigens. This triggers a series of chemical reactions that leads to an immune response, causing the body to attack the cells that are attached to that specific antigen.
If someone were to have their kidney replaced, their immune system would automatically attack the new, healthier kidney. Therefore, patients have to have their immune system suppressed to avoid these complications, but this still comes at high medical risk. People have many different self-antigens in their body. When donating an organ, the donor must match at least six of them. However, this requires careful testing; some antigen types are more uncommon than others, which means that these patients struggle more to find a matching donor.
In the case of bone marrow transplants, acute graft vs host disease (GvHD) occurs in roughly 70% of patients post-transplant. This is because, during marrow transplants, the patient’s immune system is entirely replaced with the immune system of the donor, leading to an autoimmune response directed against self-antigens, so the body begins attacking its own cells.
To avoid this, scientists have created alternative methods of synthesising tissues in the lab that are antigen-matched to the patient. This removes the need for donors and reduces immune risks. Because of the growing interest in this area, greater traction has been built towards regenerative medicine, an emerging field that focuses on engineering cells and tissues to restore normal function. Ideally, these artificial tissues would be generated entirely from human cells with highly precise and dynamic 3D construction, in order to mimic the complex environment surrounding tissues inside the body. However, early in vitro methods performed with cells taken out of the body’s internal environment were restricted to tissues on the scale of only a few millimetres, due to the limitations of current methods.
What is currently being done?
Tissues and organs are an interconnected network of cells that function together, requiring cell diversity and highly sensitive communication and transport of nutrients. The presence of blood vessels is essential for overall functioning; this is known as the vasculature of the tissue. However, this also poses a major obstacle during in-lab synthesis. It is difficult to copy the structure and function of these vessels, which means tissues do not receive enough nutrients and do not grow to a sufficiently high degree.
Conventional methods involve distributing stem or progenitor cells within a scaffold enriched with growth factors and other molecules to mimic the extracellular matrix (ECM), which is the environment within which all cells residue and grow. Stem cells are cells that can divide indefinitely and have the ability to develop into various cells from different organs or tissues, such as gut, blood or heart (cardiac) cells. The final cells developed from this process are terminally differentiated, and cells in between stem and terminal differentiation are known as progenitor cells.
As stem cells have the capability of developing into multiple different cell types, they are the most versatile making them very clinically appealing. These cells are present in many organs and can also be derived indirectly by sampling terminally differentiated cells and reprogramming them back into stem cells before use. This is a reversal of the development process, and resulting cells are known as induced pluripotent stem cells (iPSCs) as seen in Figure 1. New innovations in this field involve the use of nanopatterned surfaces to direct the spreading and differentiation of seeded cells and other molecules. However, tight control over cellular sublocations within the scaffold remains a problem.
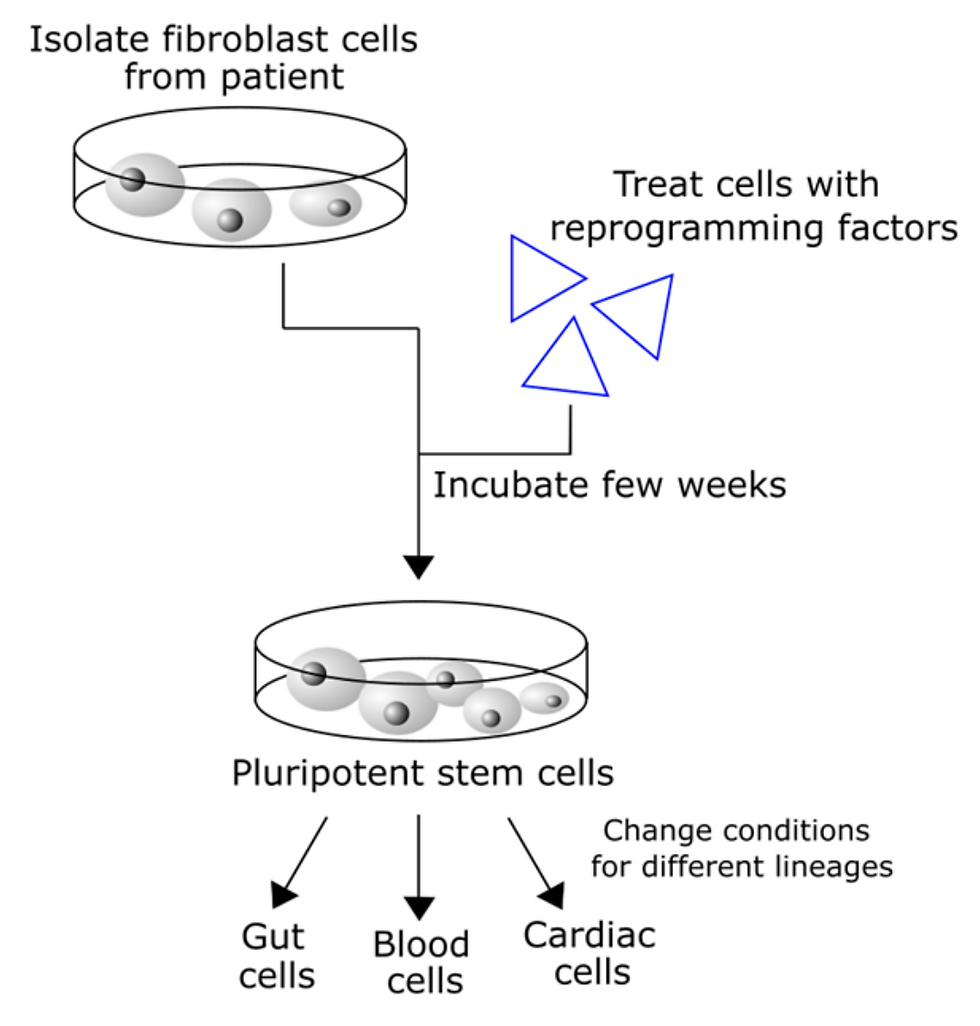
Figure 1: Diagram showing the process of re-engineering stem cells from adult tissue, to form cells of different lineages. Generating induced pluripotent stem cells (iPSCs) from adult somatic cells, a process which allows for the reengineering of tissue differentiation and underlies all regenerative medicine approaches.
How can we address the limitations of current tissue regeneration methods?
A newfound solution is to use 3D printing to generate and deposit materials in layers that can be changed to a high degree of sensitivity. This method offers several advantages as it is high throughput and reproducible. It can also be used to easily organise a mixture of cell placements within the desired structure. Previous uses of 3D printing in medicine included prosthetics, anatomy models for surgeons to practice procedures on, and dental implants; now scientists are using actual cells instead of plastics and metals to form living tissues. The procedure by which scientists do this can be broken down into the following steps:
Imaging techniques to copy internal structures
Design approach
Material and cell selection
Printing strategies
Applications of bioprinting
We will now look into these steps in more detail.
Imaging techniques to copy internal structures
As seen above, the process starts with imaging techniques that generate a blueprint of tissues with computer tomography (CT) or magnetic resonance imaging (MRI) to view internal body structures. CT scans, in particular, can combine X-ray images to generate 3D cross-sectional slices of tissues and organs. These images are then used in computer-aided design (CAD) to create a model with parameters for material and cell composition. The prototypes are converted to a series of 2D layers which are then built up to 3D structures. Printing requires the deposition of cells and other molecules layer-by-layer, which is then incubated as a complete tissue construct in a bioreactor. 3D-bioprinted structures are not only used for implantation but also can be used for in vitro drug testing to assess clinical safety and viability.
Picking the right design approach
The three main design approaches to 3D bioprinting are biomimicry, autonomous self-assembly, and mini-tissue building blocks. Biomimicry involves biologically-inspired engineering applied primarily to create new materials for industry. In 3D bioprinting, this requires the identical reproduction of the cellular environments and components of a tissue, for example, setting up physiologically accurate gradients of factors. This involves a precise understanding of the microenvironment as well as the physical forces exerted.
Autonomous self-assembly uses embryonic organ development as a guide, where self-assembling does not need a prior scaffold in place but instead begins with cellular spheroids that undergo fusion and organisation similar to how developing tissues form in embryos.
Mini-tissue building blocks are developed on the basis that all organs and tissues can be broken down into smaller units, such as nephrons in a kidney. These units can be fabricated and assembled into larger constructs as seen in “organs-on-a-chip” technologies, which are connected by a microfluidic network to screen for drugs and vaccines. Microfluidics, in this case, replace the need for vascular tissue, by providing an alternative system through which molecules can be exchanged from location to location.
These design approaches are usually integrated, as each method has its advantages and disadvantages.
Selecting materials and cells
Natural polymers like gelatin, collagen and fibrin are predominantly used in 3D printing due to their similarity to the extracellular matrix. However, synthetic polymers have the advantage of specific tailoring to ensure precise physical properties. Synthetic hydrogels, in particular, are both hydrophilic and absorbent, making them ideal for regenerative medicine. Polymers are also useful for crosslinking, where connections are made between layers on the molecular level. This allows for long-term compatibility and maintains stable mechanical properties in pores and channels to prevent collapse.
Bioprinted tissues should be allowed to remodel over time, forming different structures at specific points in tissue development. The 3D environment of cells within tissues and larger organ structures can affect cell attachment, proliferation, and shape. However, it is also incredibly difficult to recreate due to the sheer number of components. This limits the ability for synthetic tissues to reach physiological function, which is the level of function present in the body.
In many design approaches, cells are deposited onto a substrate or scaffold material made of these polymers and used to “seed” the structure. This acts as the initial cell population which will expand and develop into more organised groups, therefore acting as the “seed” from which the tissue grows. The cell types used in bioprinting are the same as in conventional organ regeneration (stem and progenitor cells) due to their ability to form different tissues.
Printing strategies
Once the design method has been decided, there are several strategies for tissue bioprinting such as inkjet, microextrusion, and laser-assisted printing. These methods must satisfy specific parameters, such as surface resolution, cell viability, and biological materials.
In inkjet printers, ink cartridges are replaced with biological materials, which are ejected in droplets via thermal or acoustic forces into predesigned patterns. In thermal printers, heat generates small air bubbles which collapse, thus creating pressure pulses that eject the biomaterial in given volumes. The localised heating of the printer lasts for only a few microseconds, which minimises damage to the cells. In piezoelectric bioprinting, a piezoelectric crystal induces a shape change and creates an acoustic wave when voltage is applied. This acoustic wave then breaks the biomaterials into droplets.

Figure 2: Two methods of inkjet-based bioprinting that propel the bioink through the micronozzle; left: heat-induced bubble formation, right: actuator produces acoustic waves.
Inkjet bioprinting is contactless, due to the ejection mechanisms of the droplets. Size, deposition rates, and droplet position can all be specified to create patterns and chemical concentration gradients, making it ideal for generating irregular 3D structures like branches or tubular constructs. However, this also leads to some limitations, such as only using solutions with low viscosity (an upper limit of 0.1 Pa/s) to avoid clogging during droplet formation. Thus, inkjet has only been used to form smaller scaffolds.
Extrusion bioprinting involves the generation of line structures where biomaterial is released continuously, driven by mechanical pistons. The tissue is built up in filaments instead, which leads to higher structural integrity despite having lower resolution when compared to other methods. This patterning strategy involves the use of stress forces, which can lead to cell deformation.
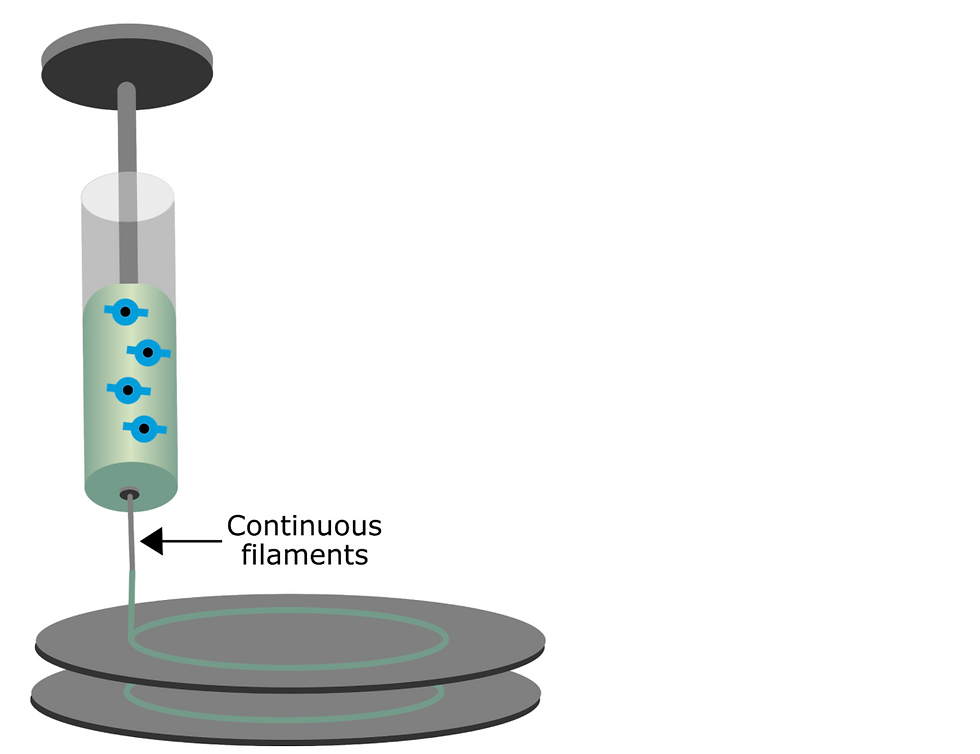
Figure 3: Extrusion-based bioprinting: a micronozzle generates continuous filaments that are layered onto a predetermined substrate.
Laser-assisted bioprinting involves a pulsed beam that is focused on a ribbon of glass covered in an energy-absorbing layer of gold or titanium and a layer of cells prepared in liquid solutions. The laser then generates a high-pressure bubble that moves materials towards the collector substrate, as shown by the grey discs in Figure 3. In some experiments, this substrate is made from quartz crystal. An example of this is in a 2018 study of UV-laser printing with a highly focused beam and a precursor hydrogel that encapsulated human umbilical vein endothelial cells (HUVECs).
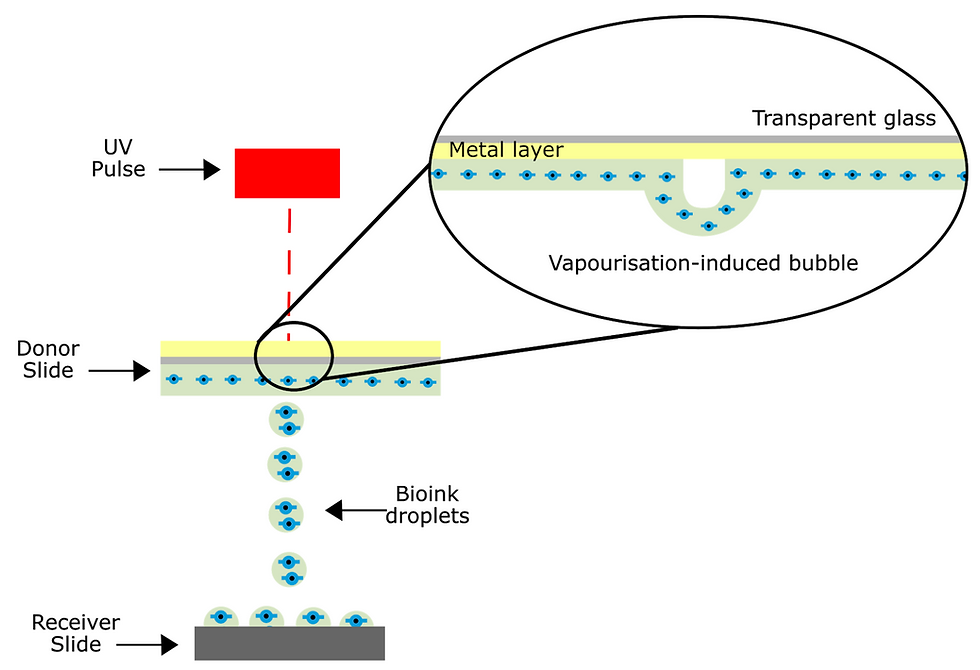
Figure 4: Laser-assisted bioprinting induces bubble formation which propels bioink droplets towards the substrate. Due to minimal interaction, this technique ensures high cell viability.
Applications of bioprinting
Although this is still a point of contention in the 3D bioprinting field, major breakthroughs have been made towards the remodelling of larger, more complex issues. One such effort was conducted at the Harvard Wyss Institute, where vascularised tissues were created at a thickness 10-fold greater than that of previous methods.
Tissues were initially seeded by combining the autonomous self-assembly approach with a novel inkjet printing technique. Hundreds of thousands of organoid building blocks (OBBs) were derived from induced pluripotent stem cells, cells which can be taken from the adult tissue of a patient (thus ensuring antigen match) and engineered to restore its ability to differentiate into a greater number of tissue types. From this, embryonic bodies, organoids, and multicellular spheroids were placed into a printed silicone mould that reinforced the initial seeding of the tissue onto a chip; the building blocks were compacted into a matrix.
Vascular channels containing living endothelial cells, which are cells that line blood vessels in the body, were printed into the mould using an inkjet printing method called sacrificial inking. The ink was patterned into the matrix and later removed, leaving behind porous channels that could branch throughout the structure similar to blood vessel networks. A second, self-supporting ink consisting of live mesenchymal stem cells (MSCs) from which skeletal tissues arise was then injected into the vascular channels.
After patterning was completed by the printer, a separate fluid composed of ECM and fibroblasts was used to fill the remaining spaces within the construct. As this fluid contained key components of the ECM itself, it provided a crosslinking stabiliser that acted akin to connective tissue throughout the structure. The result was then saturated with various nutrients and differentiation factors via an inlet-outlet system imprinted into the chip at the base of the tissue mould to ensure cell maturation. This new technique was proven to generate cardiac tissue that beat spontaneously with propagating calcium ion waves after a week of culturing.
The future of 3D bioprinting
Future developments of 3D bioprinting may focus on the combination of various printing methods such as extrusion-based and inkjet printing, all of which have their advantages and disadvantages. Design techniques will need to be tailored to specific requirements and tissue properties. Steps have been taken towards introducing vasculature into tissue constructs, a process that is essential to avoid cell death, but there are still limitations. Current protocols for OBBs derived from iPSCs are lacking in cell maturation, leading to weak function that is not stable enough to perform at physiological levels.
3D bioprinting presents a new frontier of tissue engineering that could provide an alternative to life-threatening graft procedures. In vitro applications have high-throughput methods and may even replace animal studies for drug screening. However, despite its potential, 3D bioprinting faces many limitations in the types of tissues that can be synthesised and the extent to which they can retain function over time. Further research must be conducted into optimising this process, but this field is emerging as one of the most promising areas of transplant research today.
Author: Lucy Chen, BSc Biochemistry