Cellular Respiration: Explained
- Yujean Kim
- Sep 29, 2020
- 9 min read
Updated: Jul 15, 2023
When we run a marathon, our leg muscles contract to keep our legs moving. When we eat dinner, our intestines contract to undergo peristalsis. All of these fundamental processes that occur in our body are able to function due to this metabolic process, cellular respiration.
What is cellular respiration?
Cellular respiration is the controlled release of energy from organic compounds to produce ATP (adenosine triphosphate).
It is extremely important to produce ATP in our body, as ATP is a high energy molecule that functions as an immediate source of energy or power for cellular processes. As illustrated in Figure 1, ATP is a molecule with adenosine covalently bonded to three phosphate groups. The bond that links the phosphate groups together stores a great amount of energy. Therefore, when ATP is hydrolyzed into ADP (adenosine diphosphate) and inorganic phosphate (Pi), the energy stored is released and can be used for many cellular processes. In general, cellular respiration can release energy from organic compounds to produce ATP in two ways, namely anaerobic respiration and aerobic respiration.
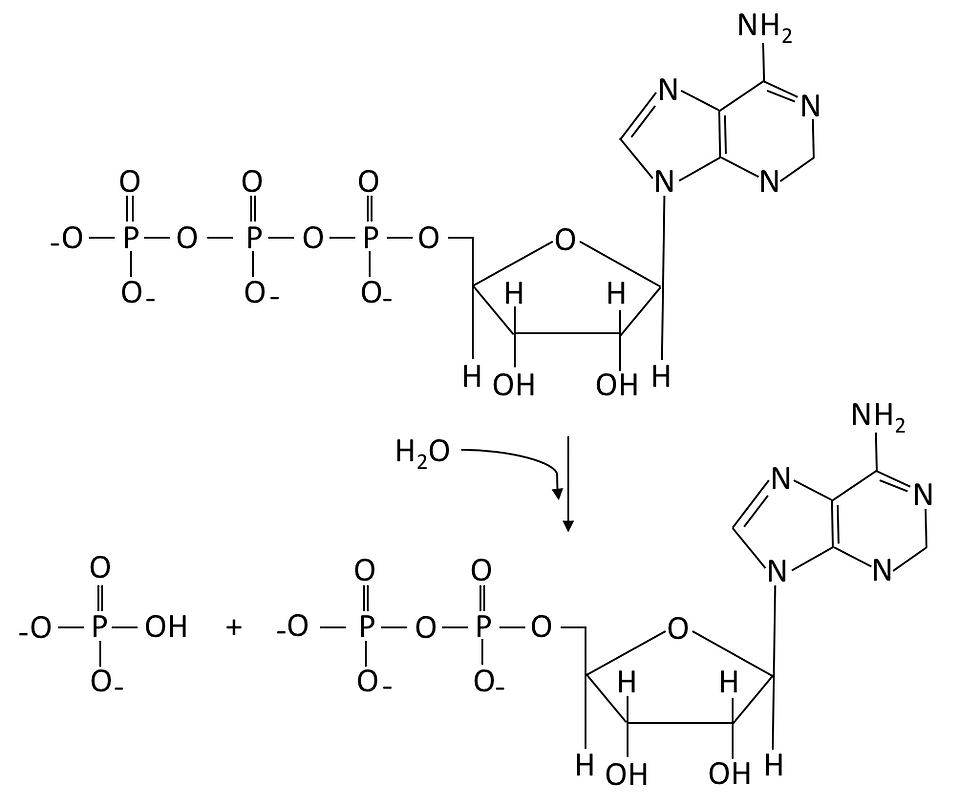
Figure 1: ATP undergoes hydrolysis reaction (i.e. using water to break chemical bonds) to produce ADP, hydrogen ion and a phosphate group (Pi).
Oxidation and reduction
During both aerobic and anaerobic respiration, two reactions occur throughout the entire process: oxidation and reduction.
In chemistry, we define oxidation as the loss of electrons, whilst reduction would be the gain of electrons. In a cell, oxidation and reduction always occur together since there is a transfer of electrons from one substance to another. Electron carriers are the small organic molecules that accept or give up electrons as required. In the context of cellular respiration, the electron carrier that is involved would be the NAD+ molecule. Since respiration is an oxidation process, NAD+ often undergo reduction by:

Since H+ ions carry one electron, accepting a H+ ion will ‘reduce’ the molecule, whereas losing a H+ ion will ‘oxidize’ the molecule. The NADH must then be recycled back to NAD+ by a cheap (because it is abundant) terminal electron acceptor since NAD+ is an expensive cofactor (meaning that there is a limited amount of NAD+ in our body). Such terminal electron acceptors include oxygen, nitrate (NO3-) and pyruvate.
Glycolysis
The very first step that takes place during cellular respiration is glycolysis. Simply put, glycolysis is the splitting of glucose into two to produce pyruvate. This can be showcased using the chemical equation below:

However, this simple definition is just the tip of the iceberg.
Glycolysis actually comprises of many complex intermediate steps, each of which are regulated by many enzymes. These enzymes are, in turn, regulated through the help of kinase and phosphorylase. Kinases are enzymes that phosphorylate molecules using organic molecules such as ATP. They often add a phosphate group to serine, threonine or tyrosine to activate the enzyme, whereby increasing the enzyme’s activity. Phosphorylases can also phosphorylate molecules, but they do so using inorganic molecules.
The glycolysis pathway is split into three processes: preparatory, cleavage and the pay-off step (see Figure 3).
The preparatory step starts off by converting carbohydrate into glucose 6-phosphate (G6P). Carbohydrates are obtained from many sources, such as glucose (food), glycogen (liver) or starch (amyloplasts). Hexokinase first phosphorylates glucose into G6P, whereas glycogen phosphorylase phosphorylates glycogen into G6P. Hexose-P-isomerase then catalyzes conversion of G6P to fructose 6-phosphate (F6P). Finally, F6P is phosphorylated using ATP to create fructose-1,6-bisphosphate (FBP) by the enzyme phospho-fructokinase. This ends the preparatory step of the glycolysis pathway.
Fructose-1,6-bisphosphate then enters the cleavage pathway. During the cleavage pathway, the 6-carbon molecules, fructose-1,6-bisphosphate, is split into two 3-carbon molecules: GAP (glyceraldehyde phosphate) and DHAP (dihydroxyacetone phosphate). DHAP is then converted into GAP in a reaction catalysed by triose phosphate isomerase. This ultimately leads GAP into the pay-off stage. This consists of many intermediate steps that are catalysed by many different enzymes (as seen in Figure 2).

Figure 2: Intermediate reactions that occur during the pay-off stage of the glycolysis reaction.
Overall, glycolysis produces a net yield of 2 ATP and 2 NADH. Once pyruvate is produced, it is destined to participate in two further processes: aerobic oxidation and anaerobic respiration.

Figure 3: The glycolysis pathway is split into three stages: preparatory, cleavage and pay-off stage. The preparatory step converts glucose into fructose-1,6-bisphosphate. The cleavage step then splits the 6-carbon fructose-1,6-bisphosphate into two 3-carbon molecules. Ultimately the pay-off step converts GAP into pyruvate.
Anaerobic respiration
Once glycolysis finishes, pyruvate will undergo anaerobic respiration (“anaerobic” refers to the lack of oxygen).
Anaerobic respiration is a type of cellular respiration that partially breaks down organic molecules in the cytoplasm without the use of oxygen. Because organic molecules are not completely broken down during anaerobic respiration, a small yield of ATP is produced instead. The products of anaerobic respiration vary between species. Humans undergo anaerobic homolactic fermentation, whereas yeasts and plants undergo anaerobic alcoholic fermentation.
Anaerobic homolactic fermentation is the process when one molecule of glucose is ultimately converted to two molecules of lactic acid. During anaerobic homolactic fermentation, pyruvate will form lactate by oxidizing NADH to NAD+ with lactate dehydrogenase. This is because they will not be able to reduce NAD+ to NADH due to the absence of a terminal electron acceptor (namely oxygen). Thus, NADH cannot convert back to NAD+ in a normal way. This ultimately forms to equation:

Humans often use anaerobic respiration while doing intense exercise. This is because anaerobic respiration can supply ATP rapidly for a short period of time. Consequently, this can maximize the power of muscle contraction during intense sports.
However, there is a limitation as to how much anaerobic respiration a human can use. Lactate is toxic to our body since it is acidic. A buildup of acidity in our body can disrupt many cell metabolites, thereby causing many cellular reactions to become dysfunctional. Therefore, there is a limit to the lactate concentration the body can tolerate, although the lactate threshold depends on each individual. Nonetheless, this can be solved by supplying oxygen since it breaks down lactate in our body. Henceforth, it is important to rest and catch our breath after doing an intense exercise.
Yeast and plants, on the other hand, undergo anaerobic alcoholic fermentation by breaking down glucose to produce ethanol, carbon dioxide and ATP:

Due to the yeast’s ability to respire anaerobically, they have been used to produce bread and wine for many years. Yeast is added to dough and placed in the oven when making bread. The carbon dioxide that is produced from anaerobic respiration will create bubbles of gas in the bread, which will cause it to rise up. This ultimately gives bread a lighter texture.
On a lighter note, let’s take a moment to appreciate what yeast has given us:
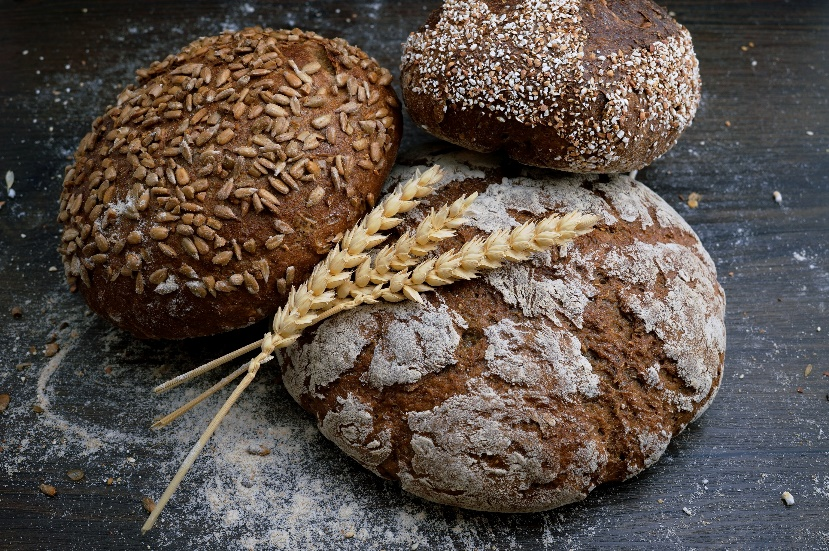
Aerobic respiration
Under aerobic conditions, pyruvate is oxidized to water and carbon dioxide via the Krebs cycle and oxidative phosphorylation.
Aerobic respiration is a type of cellular respiration that uses oxygen to oxidize an organic molecule such as glucose. Oxygen has the ability to fully break down a glucose molecule, therefore it produces a high yield of ATP. Hence, aerobic respiration can be simplified with the following equation:

Once pyruvate is produced from glycolysis, it is transferred to the matrix within the inner mitochondrial membrane as illustrated in Figure 6, where it undergoes a series of reactions collectively called the link reaction.
During the link reaction, the pyruvate will form acetyl coenzyme A (acetyl-CoA) with the help of pyruvate dehydrogenase complex. Pyruvate dehydrogenase is an extremely big enzyme – in fact it is as big as a ribosome! It plays a role in linking glycolysis to the Krebs cycle, and does this by decarboxylating pyruvate into a 2-carbon group fragment. Decarboxylation removes a carboxyl group from the pyruvate and releases carbon dioxide.
The 2-carbon group fragment then undergoes oxidation, which reduces the NAD+ molecule into NADH. This ultimately forms an acetyl group. The acetyl group finally combines with coenzyme A which produces acetyl coenzyme A. Simply put, the link reaction can be summarized into this one equation:

The acetyl coenzyme A will then enter the Krebs cycle in the mitochondrial matrix. Just like glycolysis, the Krebs cycle consists of a series of reactions supported by many different enzymes, as illustrated in Figure 2.
It all starts off with acetyl coenzyme A combining with oxaloacetate to form a citrate molecule. The citrate molecule then undergoes a series of decarboxylation and oxidation reaction to form a C4 molecule - Succinyl CoA. Following that, the C4 molecule undergoes further oxidation reactions to form oxaloacetate. The cycle then repeats by combining oxaloacetate with acetyl coenzyme A again.
Ultimately, the Krebs cycle produces 3 NADH molecules, 2 carbon dioxide molecules, 1 GTP molecule and 1 FADH2 molecule.
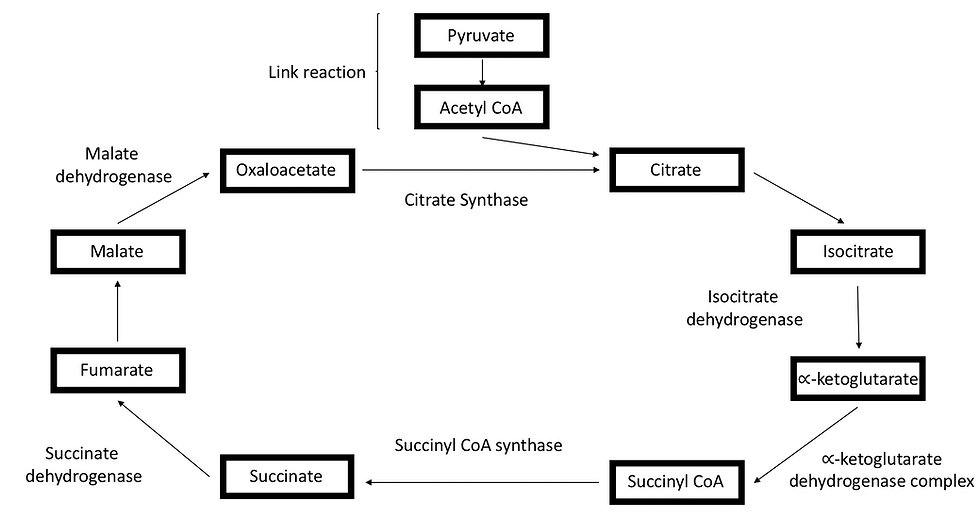
Figure 4: The link reaction decarboxylates pyruvate to produce a 2-carbon molecule. The 2-carbon molecule then combines with coenzyme A to form acetyl coenzyme A. The acetyl coenzyme A then enters the Krebs cycle by combining with oxaloacetate. After that, oxaloacetate undergoes a series of oxidation and decarboxylation reaction.
Once the Krebs cycle finishes, oxidative phosphorylation occurs. Oxidative phosphorylation takes place in the inner mitochondrial membrane and between the mitochondrial matrix and transmembrane space. Many proteins are embedded within the inner mitochondrial membrane. This includes the NADH dehydrogenase protein transmembrane complex, succinate dehydrogenase, the cytochrome-b/c complex, cytochrome-c oxidase and finally, the F-type ATP synthase.
Together these embedded proteins ultimately form an electron transport chain, as illustrated in Figure 5:
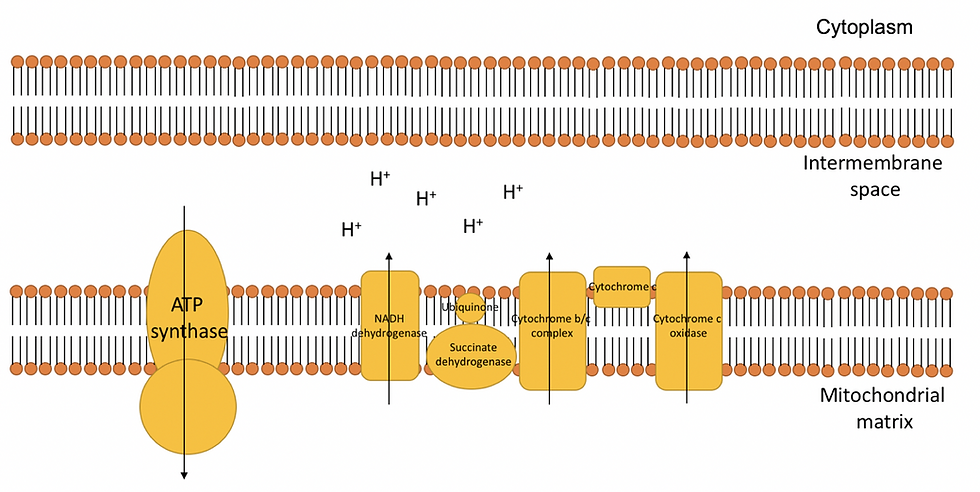
Figure 5: The electron transport chain consists of many complex proteins that are embedded within the inner mitochondrial membrane. Electrons will move from protein to protein to allow hydrogen ions to move towards the transmembrane space. This ultimately produces a difference in charge and H+ concentration across the inner mitochondrial membrane due to the high concentration of H+ ions in the intermembrane space. As a result, this forms an electrochemical gradient.
The electron transport chain all starts off in the NADH dehydrogenase complex, which is one of the largest transmembrane complexes. NADH dehydrogenase initially converts NADH to NAD+, which releases two electrons. These electrons then flow through the transmembrane complex and cause conformational changes to each of the subunits in the transmembrane. These conformational changes cause H+ ions to flow through the inner membrane and into the transmembrane space. The electrons are then transferred to ubiquinone (UQ), which is also embedded within the inner mitochondrial membrane. By accepting two electrons, the ubiquinone gets converted to ubiquinol (UQH2).
Next to the NADH dehydrogenase complex is the succinate dehydrogenase protein, which is responsible for converting the succinate formed during the Krebs cycle into fumarate. This releases two electrons which travel through the transmembrane complex. Therefore, just like the NADH dehydrogenase, succinate dehydrogenase will convert the ubiquinone to ubiquinol by accepting two electrons. However, no H+ ions are passed given a less favorable delta G (ΔG). ΔG tells us whether or not a reaction occurs spontaneously.
Consequently, all the electrons formed in NADH dehydrogenase and succinate dehydrogenase end up in ubiquinol. Ubiquinol is a fat-soluble redox carrier dissolved in the inner membrane. Ubiquinol is formed when ubiquinone consumes two hydrogen ions and two electrons. It is important the two electrons are consumed quickly since it can form a free radical instead, known as the ubisemiquinone radical (UQH•). This is a highly reactive molecule that can cause damage to the membrane (by making membranes rancid). They must be handled since it can form superoxide radical (O2.-), hydrogen peroxide (H2O2) and hydroxyl radical (OH·).
Next to succinate dehydrogenase are the cytochrome-b/c complex and cytochrome-c oxidase. They also cause H+ ions to flow across the inner membrane into the transmembrane space.
The electron transport chain will move electrons from carrier to carrier where energy is used to transfer protons across the mitochondrial matrix to intermembrane space. This will accumulate protons in the intermembrane space which will create an electrochemical gradient. While this occurs, de-energized electrons in the cytochrome-c oxidase will be accepted by oxygen molecules to form H2O. This maintains an electrochemical gradient and keeps the electron transport chain flow going, allowing more electrons to flow along the electron transport chain and NAD+/FADH to be regenerated. The oxygen also maintains the hydrogen gradient by absorbing H+ ions in the mitochondrial matrix.
Finally, hydrogen ions that accumulate in the intermembrane space will diffuse across the ATP synthase, moving down the electrochemical gradient. The energy released will phosphorylate ADP. Consequently, a high yield of ATP is formed and concludes the process of aerobic respiration.
To help you visualise the process of cellular respiration better, check out the links below:
Mitochondria
Aerobic respiration mostly occurs within the mitochondria. The mitochondria have several adaptations that bolsters the process of aerobic respiration.
Firstly, due to the presence of folded inner membranes, as illustrated in Figure 6, they have a large surface area-to-volume ratio. This allows them to increase their electron transport chain and increase their uptake/release of materials. Secondly, the mitochondria’s narrow intermembrane space ensures that a proton gradient is easily generated. Finally, the mitochondrial matrix contains many enzymes which promotes the rapid reaction of the Krebs cycle.
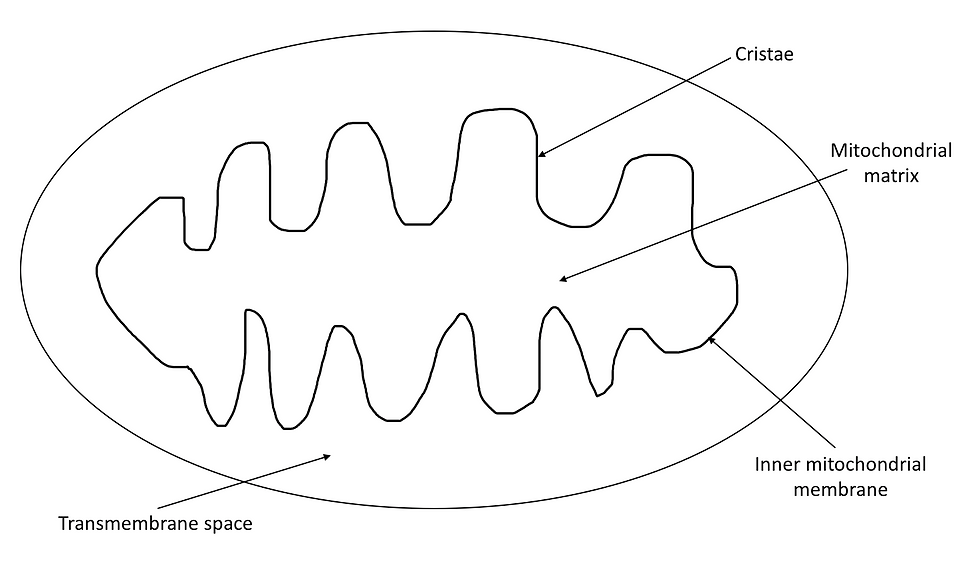
Figure 6: The structure of the mitochondria.
In conclusion, cellular respiration is a metabolic reaction that is way more complex than it may seem. However, due to this one magnificent cellular reaction, we are able to carry out all fundamental processes that occur in our body!
If you want to learn more about the process of cell respiration, check out these two books below:
Chemistry for the Biosciences Second edition by Jonathan Crowe and Tony Bradshaw
Biochemistry Fourth edition by Donald Voet and Judith G. Voet
Author: Yujean Kim, BSc Biochemistry