Living light
- Jeannine Coelho
- Dec 27, 2020
- 9 min read
Updated: Jul 15, 2023
You’ve probably heard about fireflies, but have you ever wondered why they glow? Or why jellyfish produce light even though they live in the deep dark trenches of the oceans?
The answer lies in the simple yet illuminating (pun definitely intended) phenomenon known as bioluminescence.
On that note, this article shall ‘enlighten’ you on the mechanism of bioluminescence, the diverse purposes it serves for different organisms, and how scientists have exploited it to redefine biotechnology research methods.
Let’s begin.
What is bioluminescence?
Bioluminescence is the emission of light by an organism as a result of a biochemical reaction. Meanwhile, bioluminescent organisms span across the vast spectrum of life, including both prokaryotes and eukaryotes, with bacteria, jellyfish, shrimp, and even the common glow-worm being examples of such species.
The purpose of bioluminescence
Bioluminescent organisms exhibit an assortment of emission patterns, ranging from a continuous glow to single flashes to pulses.
The core reason for this is to increase their natural fitness (i.e. how well an organism is adapted to its environment and able to reproduce), whether it’s to ward off predators, lure their prey, or even courting mates.
Let’s explore some examples:
Predator evasion
Counter-illumination
The Abraliopsis squid harbours its light-generating organs on the underside of its body. Its bioluminescence pattern mimics the sky above, thereby allowing it to appear invisible to its predators below.
Aposematism is a defense strategy where an organism makes itself look more threatening than it actually is to predators. The clusterwink snail, native to New Zealand, emits short flashes of blue-green light through its opaque shell. These light pulses can be as short as 1/50th of a second or as long as a few seconds, which is effective enough to scare away predators, and even attract their predators’ predators!
Escape
The tomopteris sea worm, upon being disturbed, flashes glowing yellow sparks from its foot-like appendages known as parapodia. While its predator is startled, the sea worm makes its escape.
Another excellent example would be the vampire squid, which is neither an octopus nor a squid (it is a cephalopod, i.e. a member of the molluscan family). Given that it lives in dark waters, its ink sacs aren’t used for an “emergency blackout”. Instead, it uses the opposite logic, whereby it shoots a dense cloud of bioluminescent mucus to surprise its attackers and give them a chance to escape. Apart from this, the vampire squid can also turn itself inside out to protect itself.
Luring prey
The anglerfish is a great example of this, in which it lures its prey by shining light through a bulb located at the top of its head (picture the scene in Finding Nemo when Dory is attracted to the light of the anglerfish).
In particular, the producer of this light is the bioluminescent bacteria, which exist in a symbiotic relationship with the angler fish. Symbiosis is a relationship between two organisms in which at least one organism benefits from coexisting with the other. In this case, the bacteria have lost genes that would enable them to live freely in the water. However, living within the angler fish's bulb provides space for the bacteria to populate and gain nutrients whenever the anglerfish feeds on its prey. In return, the bacteria produce the bioluminescent light that lures prey to the anglerfish.
Fireflies are also another exciting organism to study for bioluminescence - in that their emission patterns change at unique developmental stages and serve different purposes.
At the larval stage, the bioluminescence is emitted as short glows during the night, which acts as a form of warning to their predators. Additionally, adult fireflies have emission patterns that are species- and sex-specific, which they use to identify members of their species and potential mates. Other than that, the high flash rates and increased flash intensity in males denote higher sexual fitness to females, who in turn are required to choose their mating partner.
The mechanism of bioluminescence
Unlike biofluorescence (where organisms absorb light and re-emit it) and phosphorescence (where absorbed light is re-emitted gradually), bioluminescent reactions do not require light as an initial substrate (i.e. a molecule that is catalysed by an enzyme).
Instead, the substrate is a protein known as luciferin, in which the host organism is able to produce itself or acquire through feeding on prey. For instance, fireflies are able to produce their own luciferin, whilst certain jellyfish species feed on luciferin-producing crustaceans.
Most bioluminescent organisms produce light when luciferin reacts with oxygen. This biochemical reaction is catalysed by the enzyme luciferase. However, luciferase requires a few co-factors (i.e. molecules that an enzyme needs to perform its catalytic activity).
In particular, NADPH is first required as a cofactor that reduces luciferin (donates electrons to it). In its reduced form, luciferin is then oxygenated and converted to oxyluciferin and water by luciferase.
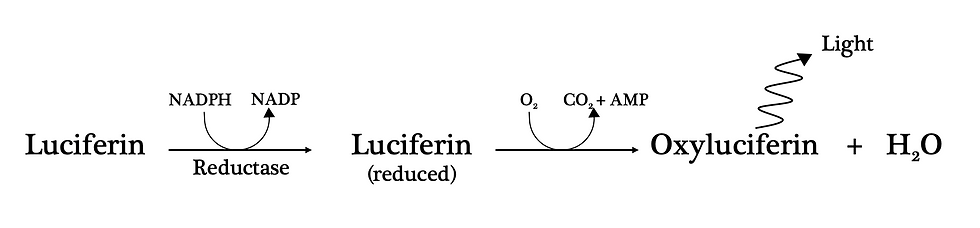
Figure 1: The biochemical reaction of fungal bioluminescence.
It should be noted that luciferases and luciferins have very different chemical structures depending on the organism. And consequently, this influences the biochemical reaction that generates the light.
For instance, in some systems, special luciferases bind and stabilise oxygenated luciferin, only allowing light emission in the presence of cations (positively charged ions) like Mg^(2+) or Ca^(2+). This selective emission acts as a sensitivity check because it permits the organism to control the timing and brightness of the light. This is of importance to the organism when conducting specific mating rituals or intimidating predators by using specific light patterns.
The bioluminescence spectrum
Bioluminescent light is generally emitted between wavelengths of 400nm to 720nm (violet to near-infrared). Specifically, the wavelength of emission is largely dependent on the environment in which the organism lives:
Deep-sea: 420nm-500m (violet to blue)
Shallow sea: 460nm-520nm (blue-green)
Land: 520nm-580nm (green-yellow)

Figure 2. Bioluminescent organisms emit light across the visible light spectrum. Adapted from ThoughtCo.
Nevertheless, it is important for you to note that the colour of this light is actually influenced by a variety of factors (i.e. not just the environment). As previously discussed, the distinct chemical structure and conformation of luciferins and luciferases is one such determinant. Meanwhile, other systems, like biofluorescence, may also interact with bioluminescence. For instance, a green fluorescent protein (GFP) can act as a secondary emitter that alters the emitted light. Besides that, anatomical structures such as the jelly-like membranes of marine organisms could also come into play, whereby they could act as biological filters that either reflects or refracts the light.
Bioluminescence lighting up the path of research
Scientific breakthroughs in the applications of bioluminescence have revolutionised research methodologies in molecular biology.
Specifically, bioluminescence is now adopted to conduct cell-based assays and imaging techniques. An assay is an investigative process to qualitatively or quantitatively measure the presence, amount, or functional activity of a target molecule or gene. On the other hand, imaging is a diagnostic method that is based on the observation of cells or molecular structures.
Below we discuss some of the assays and imaging techniques that have exploited bioluminescence.
Luciferase reporter assay
Luciferase reporter assays are generally used in cell and molecular biology to detect gene expression at the transcriptional level. In particular, it is used to detect whether a protein can activate or repress the expression of a target gene. The assay is meant to show the protein concentration and activity by the amount of light being emitted by cells that contain the protein.
This assay is highly popular given that it is convenient, quick, and cheaper than other assays such as chloroamphenicol acetyltransferase (CAT) assays (an assay that measures promoter expression), which require multiple steps and are laborious. In bioluminescence, almost all energy is converted to light, with very little being lost as heat, making this a highly accurate reaction. This is absolutely essential for scientists to determine even the smallest of changes in the transcription process.
In addition, bioluminescence is preferred over fluorescent imaging for multiple reasons. In fluorescent imaging, external illumination is required by fluorescent reporters to emit light. This is because these fluorescent reporters cannot be illuminated repeatedly or otherwise, they get bleached (their emitted light signal gets weaker with each illumination). Secondly, constantly subjecting cells to illuminations can lead to phototoxic damage. Finally, illuminating light-sensitive cells and tissue, like the retina, preventing the exact physiology of the cells being imaged.
Bioluminescence imaging, on the other hand, uses bioluminescent reporters that do not need external illumination, thereby circumventing issues of reporter bleaching, phototoxicity, and tissue-specificity. Additionally, it is a quantitative method.
How it works
The luciferase reporter assay exploits the natural bioluminescence reaction, which involves luciferase catalysing luciferin to release oxyluciferin and emit light as a by-product.
To start off, you will need two DNA plasmids (small, circular, double-stranded DNA molecules), one to express the protein that is hypothesised to influence gene transcription, and the second one acting as the expression vector (i.e. a vehicle that carries the genes of interest into a cell). On the second plasmid, the regulatory region (or promoter) of the gene of interest (GOI) is cloned upstream of the luciferase gene (check out Figure 3).
The cells are then transfected with the protein plasmid and expression vector and are allowed to undergo transcription for a certain period of time. After this, the cells are collected and lysed to release all the proteins, which will include luciferase. Luciferin is added, along with the necessary cofactors, and the enzymatic activity of luciferase is measured using a luminometer (a machine that measures the light emission from standard samples and that from cells and then translates this to a quantitative measure of luciferase expression). By fusing the GOI to the luciferase reporter gene, luciferase activity is directly correlated to the protein encoded by the GOI.
If the protein activates gene transcription, high concentrations of luciferase will be transcribed and thus, many luciferin molecules will be converted to light, giving off a bright signal (Figure 3a). If the protein represses transcriptional activity, few to no luciferase molecules are expressed, less luciferin is converted to light and there will be a weak signal measure by the luminometer (Figure 3b).
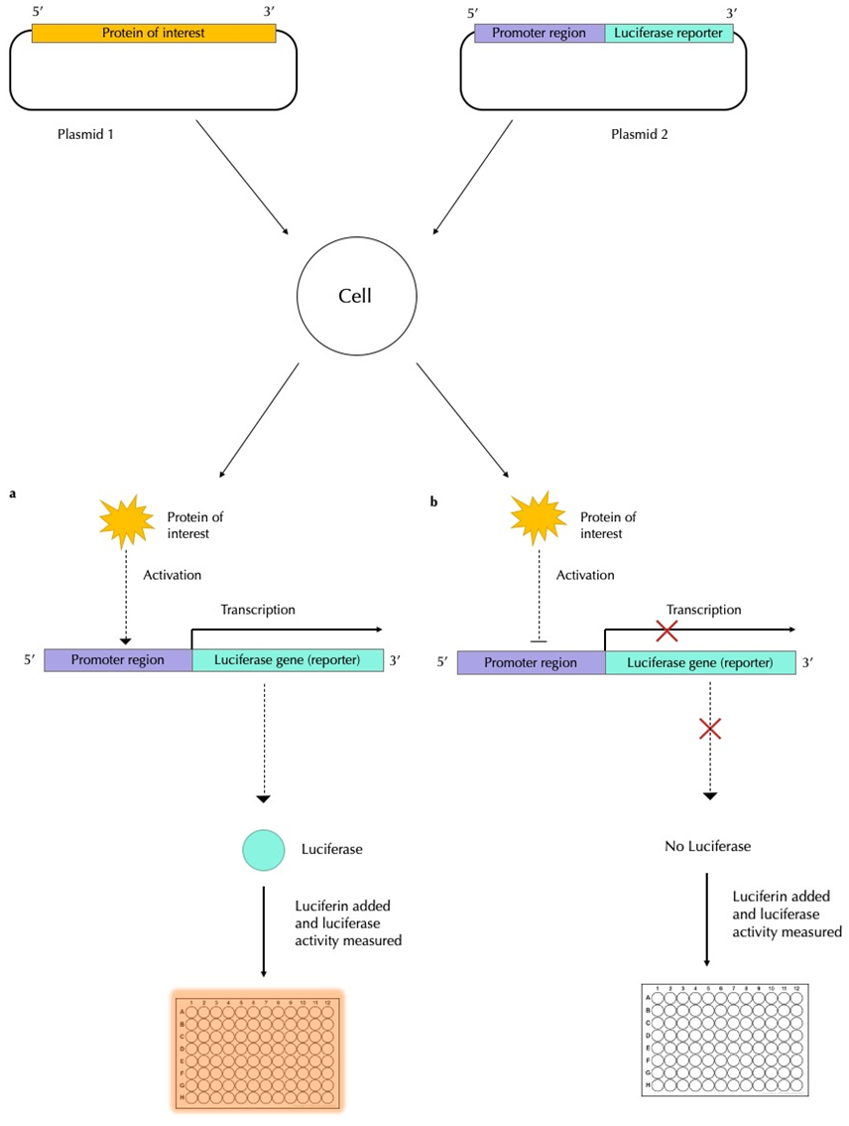
Figure 3: The bioluminescent activity of a protein that regulates gene expression. The protein of interest is copied onto a DNA plasmid. On a second plasmid, the gene of interest is cloned with the luciferase gene. These two plasmids are transfected into a cell and the cell is allowed to undergo a few cycles of transcription to allow the protein to be synthesised. Luciferin is then added to the cell culture. If the protein (a) activates gene expression, it will allow the promoter to be transcribed along with luciferase. This, in turn, catalyses luciferin to emit light, which is then measured. If the protein (b) represses gene expression, then the promoter and luciferase are not transcribed, and so luciferin is not acted upon luciferase. As a result, no light is emitted.
In experiments where multiple gene-protein functional relationships are being investigated simultaneously in the single-cell extracts, researchers use a multi-colour system, where luciferases that emit different colours are used.
Bioluminescence imaging
This technique is used to image cells in order to understand, for instance, how cancer cells grow their activity, and how they respond to drugs inside an organism.
In vivo bioluminescence imaging
In vivo bioluminescence imaging is used to observe whole living organisms in order to understand cell activity in real-time and in a more realistic set-up compared to cells in a Petri dish.
The luciferase gene is first transfected in a stable cell line (a cell line that replicates indefinitely and can express large amounts of the protein of interest) and is later established as a luciferase-expressing stable cell line (Figure 4). These cells are injected in small animals (e.g. mice) and the whole animal is imaged at different times. Moving forward, the number of cells and their distribution is compared over time to understand how the speed at which cells divide and spread across the organism. In this way, cancer cell proliferation and metastasis can be observed.
Alternatively, a luciferase protein probe is used to detect disease antigens on cell surfaces.
To design a probe, scientists prepare a gene sequence that encodes luciferase combined with an antibody (a molecule that targets and binds to a particular protein). After that, the combined luciferase and antibody probe is purified and injected into a mouse. A day later, luciferin is introduced into the mouse and the images are obtained using a charged coupled device (CCD), a highly sensitive photon detector that can track the bioluminescence within the mouse. By experimenting with different types of luciferins and luciferases, scientists are thus improving imaging techniques.
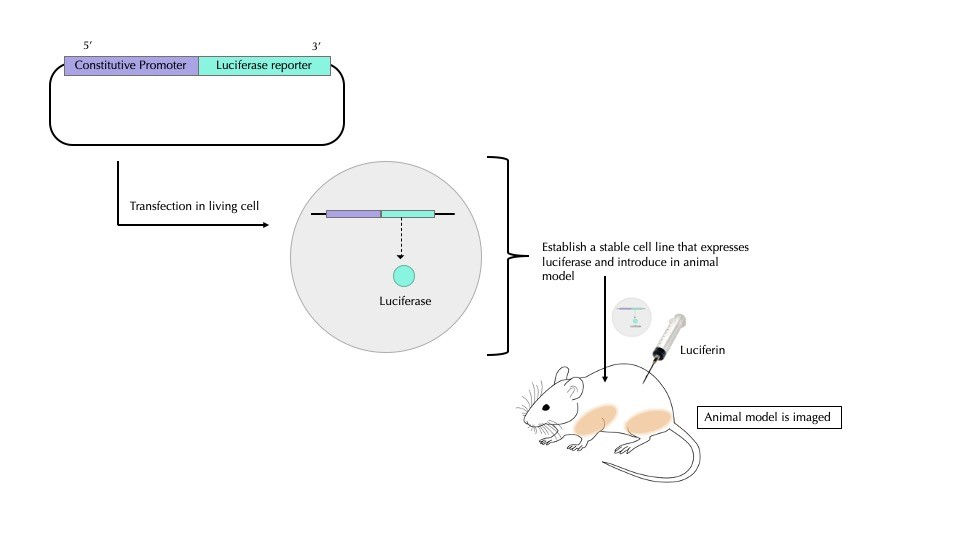
Figure 4: Conventional in vivo bioluminescence imaging involves creating a stable cell line that keeps on producing luciferase. This cell line is introduced into a specific part of the animal model (in this case, a mouse). The luciferase-expressing cells are allowed to proliferate over a few days before luciferin is injected into the mouse. The whole animal is then viewed under special cameras, a charged coupled device (CCD), that can capture the luminescence emitted. In essence, viewing the mouse at different times can reveal luminescence patterns that implicate changes in cell proliferation. This figure was adapted from Photobiology.
Some final notes
Hopefully, this article has illuminated the wonders of bioluminescence, its ubiquity in nature, and how scientists have used it to redefine research.
From its original use as survival tactics in the natural world to now an essential imaging technique yielding novel insights on cancer progression, it is clear that bioluminescence might just be one of the best examples of how scientists have translated their inspiration from nature into novel biological innovation.
Nonetheless, there are questions that are yet to be answered.
While the existence of bioluminescence has been observed in a variety of organisms, still for a lot of them, the mechanism behind the light is still a mystery. Additionally, scientists have yet to understand why some organisms, like earthworms, possess this property.
Not to mention, understanding how organisms manufacture their own light could potentially benefit our society. For instance, bioluminescence is essentially a renewable source of light, and it could theoretically replace lights that are powered by batteries and other unsustainable sources. Meanwhile, bioluminescence could be used to engineer plants that produce their own light, and these could be used in place of streetlights and thus cut the cost of running electricity throughout the night.
Overall, researching this living light could potentially answer some of nature’s enigmas, and in turn, also help us to accelerate biomedical research and design sustainable and innovative solutions to societal problems.
Some good stuff for you
If you are interested in this topic and want to learn more about bioluminescence, here are a few links describing research in this area and as well as video footage of bioluminescent organisms:
Author
Jeannine Coelho
BSc Biological Sciences with a Year in Industry
Imperial College London